27 Proteins
What are proteins?
Proteins are organic compounds made up of different building blocks (basic units) called amino acids joined together by peptide bonds (Figure 5.17). A dipeptide contains one peptide bond and two amino acids, whereas a tripeptide contains three amino acids and two peptide bonds. A peptide with more than ten amino acids is called a polypeptide. Proteins are essentially large polypeptides. The structure of a protein is determined first by the sequence of individual amino acids it has in the polypeptide chain. This is also called the primary structure of the protein.
Protein functions
- Body proteins (for example, muscle, hair, hooves, skin)
- Blood proteins (for example, albumin, globulin)
- Tissue proteins (for example, collagen, keratin)
- Enzymes and hormones
- Immune system antibodies and other peptide growth factors
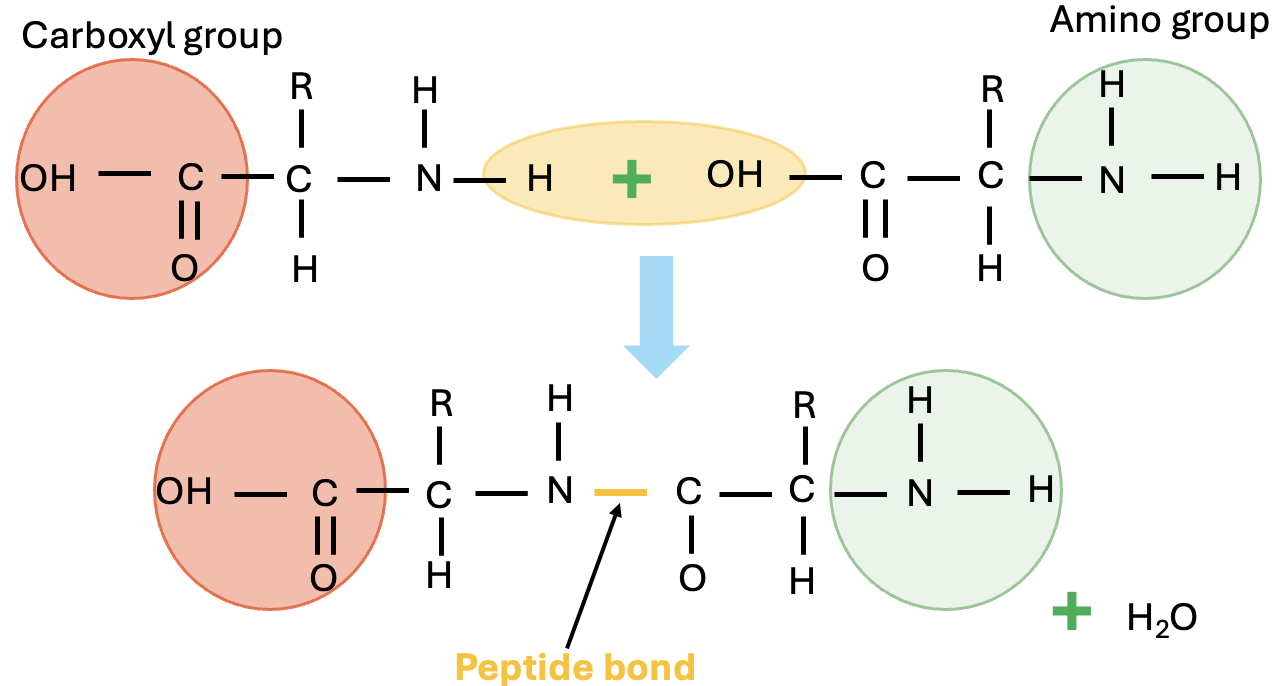
Proteins are vital for life and are the major structural components of animal tissues (for example, skin, muscles, wool, feather, tendons, eggs). In addition, proteins are also involved in biochemical (for example, enzymes), immunological (for example, immunoglobulins), transportation (for example, lipoproteins), and other regulatory (for example, hormones) activities. Proteins can also provide energy when needed.
Many of the structures in animal tissue (for example, muscle) and metabolic reactions (for example, enzymes, hormones) are catalysed by proteins. Therefore, protein synthesis is essential for maintaining life process. Provision of adequate dietary protein and amino acids are essential for maintaining growth, health, and productivity in food-producing animals. Intestinal microflora can synthesise proteins from nonprotein sources in ruminant animals.
Protein requirements vary with life stages and are high during phases of fast growth in young animals and during pregnancy and lactation. Like other macronutrients, proteins contain carbon, oxygen, and hydrogen. In addition, proteins also contain nitrogen and sulphur (in some amino acids). It is the nitrogen that makes proteins very unique in animal nutrition with respect to its digestibility, metabolism, and disposal within the animal body.
Dive deeper
Watch this video on proteins: RCSBProteinDataBank. (2017, November 21). What is a protein? [YouTube, 6:57mins]
Classification of proteins
Proteins can be classified based on their shape; solubility in water, salt, acid, base, or alcohol; or according to the nature of the prosthetic group.
Classification based on solubility and prosthetic group
-
- Globular proteins (soluble in water or dilute acids, bases, or alcohol):
- Albumin: water soluble; present as albumen in egg white; in blood circulation, it performs various functions (for example, as a carrier of lipids)
- Globulin: soluble in dilute neutral solutions; functions as part of the immune system in body defence (for example, immunoglobulins)
- Fibrous proteins (insoluble in water and are resistant to digestive enzymes):
- Keratins (for example, wool, hair, feather, hooves, horn)
- Collagen (can be converted to gelatin when heated; present in bone, teeth, tendons, and soft connective tissue)
- Conjugated proteins (contain other nonprotein compounds in structure), examples include:
- Lipoproteins (lipid-carrying protein)
- Haemoprotein (proteins with heme units)
- Glycoproteins (proteins with sugar)
- Nucleoprotein (proteins bound to nucleic acid)
- Globular proteins (soluble in water or dilute acids, bases, or alcohol):
These proteins have limited nutritional value but are important in biochemical, structural, and other metabolic functions. For example, feather meal is high in protein (keratin) but very low in digestibility and is of limited use in animal nutrition as a feed ingredient. Amino acids in the polypeptide chain in feather meal form disulfide bonds (-S-S-), which twist the polypeptide chain into a specific coiled structure such as helix or sheet. This is called a secondary structure. These bonds account for the tough physical properties of hooves and horns and their low digestibility.
The disruption of secondary structure by heat treatment causes denaturation of the proteins (for example, egg white coagulation during cooking). Certain anti-nutritional factors in feed (for example, trypsin inhibitor in soybean meal) are proteins. Heat processing denatures trypsin inhibitor in soybean meal and can enhance digestibility.
Protein structure
A protein’s shape is critical to its function. For example, an enzyme can bind to a specific substrate at an active site. If this active site is altered because of local changes or changes in overall protein structure, the enzyme may be unable to bind to the substrate. To understand how the protein gets its final shape or conformation, we need to understand the four levels of protein structure: primary, secondary, tertiary, and quaternary.
Primary structure
Amino acids’ unique sequence in a polypeptide chain is its primary structure. For example, the pancreatic hormone insulin has two polypeptide chains, A and B, and they are linked together by disulfide bonds. The N terminal amino acid of the A chain is glycine; whereas, the C terminal amino acid is asparagine(Figure 5.18). The amino acid sequences in the A and B chains are unique to insulin.
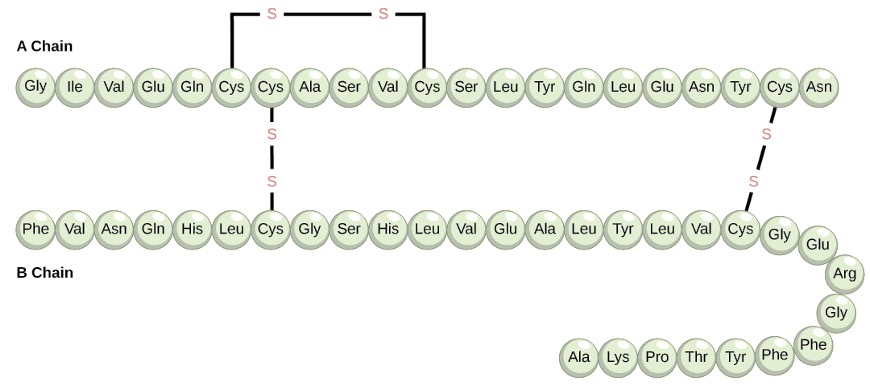
The gene encoding the protein ultimately determines the unique sequence for every protein. A change in nucleotide sequence of the gene’s coding region may lead to adding a different amino acid to the growing polypeptide chain, causing a change in protein structure and function.
Secondary structure
The local folding of the polypeptide in some regions gives rise to the secondary structure of the protein. The most common are the α-helix and β-pleated sheet structures (Figure 5.19). Both structures are held in shape by hydrogen bonds. The hydrogen bonds form between the oxygen atom in the carbonyl group in one amino acid and another amino acid that is four amino acids farther along the chain.
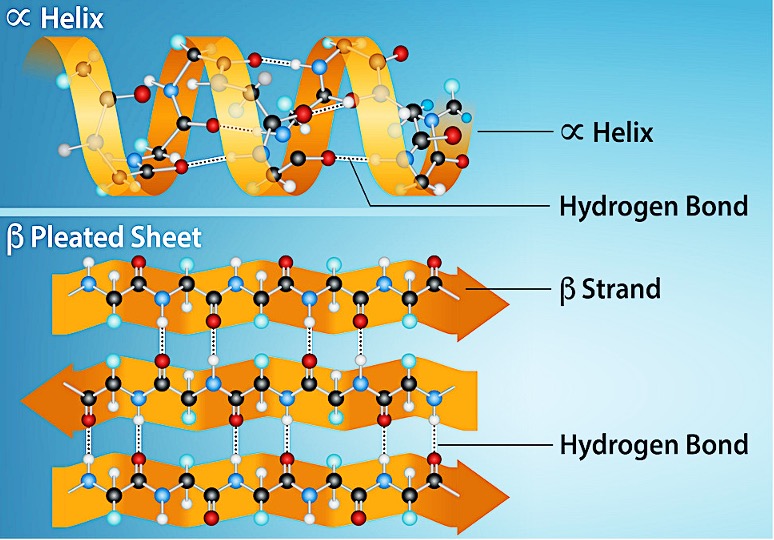
Every helical turn in an alpha helix has 3.6 amino acid residues. The polypeptide’s R groups (the variant groups) protrude out from the α-helix chain. In the β-pleated sheet, hydrogen bonding between atoms on the polypeptide chain’s backbone form the ‘pleats’. The R groups are attached to the carbons and extend above and below the pleat’s folds. The pleated segments align parallel or antiparallel to each other, and hydrogen bonds form between the partially positive hydrogen atom in the amino group and the partially negative oxygen atom in the peptide backbone’s carbonyl group. The α-helix and β-pleated sheet structures are in most globular and fibrous proteins and they play an important structural role.
Tertiary structure
The polypeptide’s unique three-dimensional structure is its tertiary structure (Figure 5.20). This structure is in part due to chemical interactions at work on the polypeptide chain. Primarily, the interactions among R groups create the protein’s complex three-dimensional tertiary structure. The nature of the R groups in the amino acids involved can counteract forming the hydrogen bonds we described for standard secondary structures. For example, R groups with like charges repel each other and those with unlike charges are attracted to each other (ionic bonds). When protein folding takes place, the nonpolar amino acids’ hydrophobic R groups lie in the protein’s interior; whereas, the hydrophilic R groups lie on the outside. Scientists also call the former interaction types hydrophobic interactions. Interaction between cysteine side chains forms disulfide linkages in the presence of oxygen, the only covalent bond that forms during protein folding.
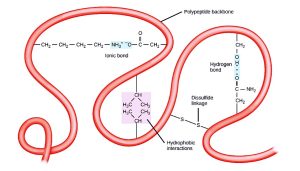
All of these interactions, weak and strong, determine the protein’s final three-dimensional shape. When a protein loses its three-dimensional shape, it may no longer be functional.
Quaternary structure
In nature, some proteins form from several polypeptides, or subunits, and the interaction of these subunits forms the quaternary structure. Weak interactions between the subunits help to stabilise the overall structure. For example, insulin (a globular protein) has a combination of hydrogen and disulfide bonds that cause it to mostly clump into a ball shape. Insulin starts out as a single polypeptide and loses some internal sequences in the presence of post-translational modification after forming the disulfide linkages that hold the remaining chains together. Silk (a fibrous protein), however, has a β-pleated sheet structure that is the result of hydrogen bonding between different chains.
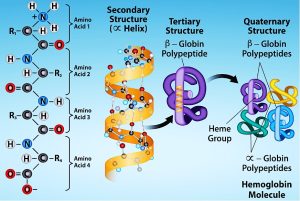
Denaturation and protein folding
Each protein has its own unique sequence and shape that chemical interactions hold together. If the protein is subject to changes in temperature, pH, or exposure to chemicals, the protein structure may change, losing its shape without losing its primary sequence in what scientists call denaturation. Denaturation is often reversible because the polypeptide’s primary structure is conserved in the process if the denaturing agent is removed, allowing the protein to resume its function. Sometimes denaturation is irreversible, leading to loss of function. One example of irreversible protein denaturation is frying an egg. The albumin protein in the liquid egg white denatures when placed in a hot pan. Not all proteins denature at high temperatures. For instance, bacteria that survive in hot springs have proteins that function at temperatures close to boiling. The stomach is also very acidic, has a low pH, and denatures proteins as part of the digestion process; however, the stomach’s digestive enzymes retain their activity under these conditions.
Protein folding is critical to its function. Scientists originally thought that the proteins themselves were responsible for the folding process. Only recently researchers discovered that often they receive assistance in the folding process from protein helpers, or chaperones (or chaperonins) that associate with the target protein during the folding process. They act by preventing polypeptide aggregation that comprise the complete protein structure, and they disassociate from the protein once the target protein is folded.
Amino acids
Amino acids are the building blocks of proteins. There are more than 300 different amino acids known to exist in nature. Out of these, about 20 amino acids are important constituents of animal proteins and are associated with muscles, connective tissues, skin, feathers, horns, blood, enzymes, and hormones. There about 10 amino acids that should be present in the diet of animals because animal tissues cannot synthesise them or cannot make the adequate amount needed for metabolic functions; these are called essential amino acids. A few other amino acids such as citrulline and ornithine do not occur in animal tissues but are involved in cellular metabolic functions.
Amino acids are the building blocks of proteins
Essential amino acids must be supplied through the diet. Animals cannot synthesise them or cannot make the adequate amount needed.
All amino acids by definition contain at least one amino group (-NH2) and one carboxyl group (–COOH) on the C atom adjacent to the carboxyl group (Figure 5.22). An exception to this is proline (imino acid), which is lacking a free amino group. The general structure of an amino acid is shown below by the amino acid glycine, the simplest of the amino acids (Figure 5.22) The R group (shown in the red circle) in amino acids varies for different amino acids. The R group is the remainder of the molecule or any other group attached to the C atom. In the case of glycine, it is an H group. The amino group (NH2) provides basic properties to the amino acid, and the carboxyl (COOH) group provides acidic properties. Amino acids important in animal nutrition are alpha (α) amino acids, which are carboxylic acids with an amino group on the α-carbon (or the first carbon attached to a functional group).
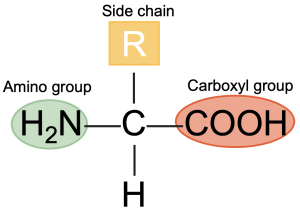
Amino acids can exist in two isomeric forms, the D- and L-isomers. The D- and L-amino acids differ in their configuration of groups around the asymmetric α-carbon. Only L-amino acids are used in protein synthesis, except methionine, where both D- and L-amino acids can be used by the animal. DL methionine is commonly used as an amino acid supplement in animal feeds.
All amino acids except glycine contain an asymmetric α-carbon (with four different chemical groups attached to it). Compounds with asymmetric carbons can exist as isomers.
Essential amino acids
Animal body can synthesise some amino acids in sufficient amounts. However, animals cannot synthesise some amino acids, or not in the amount that is needed for body requirements. Such amino acids need to be provided through diet in monogastric animals; these are called essential (indispensable) amino acids. Pigs, dogs, and humans need a total of 10, and chickens and cats need a total of 11 essential amino acids. A list of essential amino acids needed by monogastric animals is shown below.
It should be borne in mind that other nonessential amino acids are also physiologically important for metabolic functions in the body and are made from other precursors available through diet (for example, carbohydrates, nonprotein nitrogenous substances). The need for essential amino acids varies in animals. For example, horses need essential amino acids, whereas ruminant animals (for example, cattle, sheep, goats) generally do not have a requirement of essential amino acids as they are synthesised by rumen microbes.
List of essential amino acids and their common abbreviations
- Arginine (Arg)
- Histidine (His)
- Lysine (Lys)
- Isoleucine (Ile)
- Leucine (Leu)
- Methionine (Met)
- Phenylalanine (Phe)
- Threonine (Thr)
- Tryptophan (Try)
- Valine (Val)
In addition to these 10 essential amino acids, cats and chickens need the following extra amino acids.
- Chickens need glycine (Gly).
- Cats need taurine (Tau).
Digestion is the process by which ingested feed is broken down physically and chemically to simple products for absorption from the digestive tract. In the case of proteins, it involves denaturing of proteins to expose the peptide bonds, followed by hydrolysis and release of free amino acids.
Protein digestion involves the denaturing of peptide bonds and the release of free amino acids.
Protein-digesting enzymes
Protein-digesting enzymes are either endopeptidase or exopeptidase. Endopeptidases break peptide bonds within the primary structure into smaller fragments. Exopeptidases cleave amino acids off the terminal end of the protein molecule. Carboxypeptidases remove an amino acid from the end with a free carboxyl group, and aminopeptidase act on the terminal amino acid with a free amino group.
Types of protein-digesting enzymes
- Endopeptidase
- Exopeptidase
- Carboxypeptidase
- Aminopeptidase
Protein digestion
Protein digestion begins in the stomach.
Gastrin, a hormone, initiates the breakdown of proteins in the stomach. The presence of food in the stomach leads to the secretion of pepsinogen by the chief cells of the gastric mucosa. Pepsinogen is activated to form pepsin (active form) through HCl produced by parietal cells of the gastric mucosa. Pepsin is an endopeptidase. In young animals, milk-coagulating rennin is secreted into the stomach for clot formation, which aids in transport into the small intestine.
Protein-digesting enzymes, site of production, and active forms
- Pepsin (Stomach)
- Enterokinase (Duodenum)
- Trypsinogen (Pancreas, inactive) to trypsin (small intestine)
- Chymotrypsinogen (Pancreas, inactive) to chymotrypsin (small intestine) by trypsin
- Procarboxypeptidase (Pancreas, inactive) to carboxypeptidase (chymotrypsin, small intestine) by trypsin
The next portion of digestion occurs in the small intestine, which plays a major role in protein digestion. The hormone secretin, in the duodenum, stimulates enzymatic secretions from the pancreas, which includes three inactive forms: trypsinogen, chymotrypsinogen, and procarboxypeptidase. Enterokinase, also secreted at the duodenum, converts trypsinogen into trypsin, which then converts chymotrypsinogen and procarboxypeptidase to their active forms—chymotrypsin and carboxypeptidase.
Trypsin plays a very crucial role in protein digestion in the small intestine.
Digestion is finished off by other enzymes including aminopeptidases and dipeptidases from mucosal membranes. The goal of this process is to bring polypeptides down to single free amino acids.
Just like carbohydrates and fats, absorption is facilitated by the villi within the small intestine into the bloodstream. Normal free proteins are transported via active transport, energy requiring, and use sodium as a kind of cotransported molecule. Whole proteins use a direct transport method that does not require energy. Free amino acids are the major form for absorption into the circulatory system. However, some di-, tri-, and oligopeptides are also absorbed. Specific carrier proteins based on the nature of the amino acid (for example, neutral, basic, acid, large, small) are involved in amino acid transport. The naturally occurring L-forms of amino acids are absorbed preferentially to D-forms. Some amino acids may compete with others for carrier proteins and transport. For example, arginine inhibits lysine transport and high concentrations of leucine increase the need for isoleucine. Some neutral amino acids inhibit basic amino acid transport.
The fate of amino acids
Absorbed amino acids could be used for tissue protein, enzyme, and hormone synthesis and deamination or transamination, and the carbon skeleton can be used for energy. Undigested proteins in the hindgut are subjected to microbial fermentation leading to the production of ammonia and other polyamines.
Protein digestion in ruminants
Protein digestion in the ruminant animals can be divided into two phases: (i) digestion (degradation) in the reticulorumen and (ii) digestion in the abomasum and small intestine. Therefore, in ruminant animals, dietary proteins are classified as rumen degradable and rumen undegradable proteins.
In ruminants, dietary proteins can be classified as degradable or undegradable proteins.
Like monogastric animals, the main goal for protein supplementation is to provide amino acids to the animal. However, in ruminants, proteins serve as a source of nitrogen for rumen microbes so they can make their own microbial protein from scratch. Microbes do not care where the nitrogen sources come from and can use nonprotein nitrogenous substances such as urea for microbial protein synthesis. Urea is 100% degradable in the rumen by microbial urease (can be toxic at higher levels).
Protein entering the rumen may be degraded by both bacteria and protozoa, which produce proteolytic enzymes. The rumen microbes provide proteases and peptidases to cleave peptide bonds in polypeptides to release the free amino acids from proteins. Several factors such as solubility and the physical structure of protein can affect rumen degradation. These rumen-degraded amino acids release NH3 and the C skeleton by a process called deamination. Along with volatile fatty acids (from carbohydrates), rumen microbes synthesize their own microbial protein, which serves as a primary source of protein to the host ruminant animals.
Microbial protein is enough for maintenance and survival but not for high-producing animals. Ammonia absorbed from rumen is converted to urea and secreted into the blood as blood urea nitrogen (BUN). Urea can be filtered and recycled to the rumen via saliva or through the rumen wall. The concentration of BUN in ruminants reflects the efficiency of protein utilisation.
Not all proteins are degraded in the rumen.
Proteins that are not degraded by rumen microbes are called escaped, bypassed, or undegradable (rumen undegradable protein, RUP), and have a low rumen degradation rates (for example, proteins in corn).
RUP enters the abomasum and small intestine of the ruminant animal for digestion and absorption. Proteins reaching the small intestine could be RUP or those from microbial sources. The amino acid needs of the host animal are met by RUP and microbial proteins. Both ruminants and monogastrics require the essential amino acids in their diet, and amino acids cannot be stored within the body, so a constant dietary supply is necessary. Some of the similarities and differences in monogastric and ruminant animals in protein digestion or degradation are shown in table 5.1 below.
Table 5.1. Comparison of protein digestion in monogastric and ruminant animals
Monogastrics | Differences (Ruminants) |
Amino acid profile at small intestine reflects the diet | Amino acid profile at the small intestine is different from diet |
No upgrading of low quality dietary protein | Up-grade low quality dietary protein |
Protein quality not downgraded | Down-grade high quality dietary protein |
Cannot use non protein nitrogen | Able to use non protein nitrogen (for example, urea) |
Constant supply of amino acids are required | Constant supply of amino acids are required |
Case study
Optimising Protein Intake in Livestock
Livestock fed with corn-based diets showed signs of protein deficiency, such as poor growth rates, reduced milk production, and overall poor health. Testing revealed low levels of essential amino acids, particularly lysine and methionine.
Research
Among the cereal grains, corn has the highest bypass potential. However, it should be noted that corn is deficient in essential amino acids such as lysine and methionine. Different feed formulations are tested in feed trials, including corn-based diets supplemented with animal protein sources like fish meal and meat meal. Animal protein sources such as fish meal and meat meal have high bypass potential. Forages are dried and subjected to heat treatment to increase bypass potential. Feed processing methods, such as pelleting, steam rolling or flaking, tend to denature the feed protein due to the generation of heat, thereby ‘protecting’ the protein from lysis in the rumen. Rumen protected protein sources (through formaldehyde treatment) that remain intact in the rumen and dissolve in the abomasum are commercially available.
Outcome
Supplementing with animal protein sources and using heat-treated forages improves protein intake and overall livestock health. Feed processing methods and rumen-protected proteins effectively protect feed protein from lysis in the rumen, ensuring better protein absorption in the abomasum and addressing the deficiencies observed with corn-based diets.
All proteins in the body are in a state of constant flux, the size of the amino acid pool depends on a balance between synthesis and degradation. Amino acid metabolism involves protein and nonessential amino acid synthesis and disposal of toxic ammonia.
The fate of absorbed proteins
Absorbed proteins are used for anabolic purposes such as synthesis of nonessential amino acids, tissue protein synthesis, enzyme or hormone synthesis, deamination, or transamination.
Amino acid synthesis and degradation are brought about by two reactions called transamination and deamination that occur in the liver.
Synthesis of nonessential amino acids
The liver is the major site of amino acid metabolism. The liver has enzymes such as transaminases and is responsible for nonessential amino acid synthesis through a process called transamination (Figure 5.23). In this reaction, an amino group from one amino acid is transferred to an organic acid to form a new amino acid. Vitamin B6 (pyridoxine) is needed for transaminase activity.
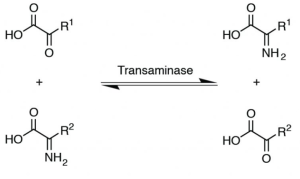
Transamination also provides a link between protein and carbohydrate metabolism, where certain amino acids can use their C skeleton for glucose synthesis. Deamination is the removal of amino groups from amino acids to form ammonia. This process is needed for getting rid of nitrogen from the animal’s body. After deamination or transamination, C skeletons are left and are used for making glucose, ketone bodies, or energy production.
Transamination is a chemical reaction that transfers an amino group to a keto acid to form new amino acids.
The fate of the carbon skeleton
- Oxidised for energy
- Used for glucose synthesis
- Used for ketone body formation
- Used for fat synthesis
All amino acids, except leucine and lysine, are glucogenic, meaning that they can use the C skeleton for glucose synthesis. Leucine and lysine are strictly ketogenic amino acids (forms ketone bodies) and can provide acetyl CoA as an energy source. Since both carbons of acetyl CoA are lost in the tricarboxylic acid (TCA) cycle, it cannot provide glucose. Some amino acids (isoleucine, phenylalanine, tyrosine, tryptophan) are both glucogenic and ketogenic.
All amino acids, except leucine and lysine, are glucogenic. Leucine and lysine are strictly ketogenic.
Some amino acids can be glucogenic or ketogenic.
Urea cycle and detoxification of ammonia
The ammonia liberated from amino acid degradation is toxic to the central nervous and needs to be excreted or detoxified. Most mammals detoxify ammonia and excrete it as urea in the urine, while birds excrete it as uric acid (a white substance in the excreta). The detoxification of ammonia to form urea is brought about by the urea cycle through two tissues (liver and kidney).
Detoxification of ammonia to urea is through the urea cycle.
Two nonprotein amino acids (amino acids not used for protein synthesis) involved in the urea cycle are ornithine and citrulline The first step in the urea cycle is the formation of carbamoyl phosphate through the condensation of ammonium ions with bicarbonate ions in the mitochondria of the liver.
Ornithine reacts with a compound called carbamoyl phosphate and forms citrulline. Citrulline is easily permeable and gets into the cytosol and reacts with aspartate, forming argininosuccinate (2 ATP needed). The enzyme argininosuccinate lyase cleaves argininosuccinate into arginine and fumarate and fumarate enters the TCA cycle. Arginine is lysed into ornithine and splits urea off, producing ornithine, to start the cycle again. Hence arginine can be a nonessential amino acid but not available for protein synthesis.
The kidneys synthesise arginine from citrulline. The liver breaks down arginine into urea and ornithine.
Poultry cannot synthesise carbamoyl phosphate and hence they cannot make urea. Instead, glutamic acid, glycine, and methionine are used for uric acid synthesis. Hence poultry need high levels of methionine, arginine, and glycine in their diet for optimum production. Since the formation of urea through the urea cycle is ATP dependent, feeding animals poor quality or excess protein is energy demanding and can lead to environmental problems (for example, air ammonia, groundwater pollution).
In the ruminant animals, rumen NH3s are sent to the liver via the portal vein and undergo the urea cycle back to urea and enter the blood, where they are eventually secreted in the urine or brought back into the digestive tract as an N source for the rumen microbes.
Protein synthesis occurs in every tissue of the body. Protein synthesis lies ultimately in the genetic code.
Briefly, nucleic acids such as deoxyribonucleic acid (DNA; molecule that stores genetic information) and ribonucleic acid (RNA) consists of nucleotides. DNA contains the genetic code of the animal and is the blueprint of protein synthesis. DNA controls the formation of RNA (a template for transcription). There are three different types of RNA (ribosomal RNA, messenger RNA, and transfer RNA). All three are involved in protein synthesis. Ribosomal RNA is the part of the structure of ribosomes, which has three base codons that code for amino acids (site of protein formation). Transfer RNA picks up specific amino acids from cytoplasm and transports it to the ribosomes (workbench where proteins are made). Transfer RNA (tRNA) acts like an adapter during protein synthesis. Each tRNA carries a specific amino acid. Messenger RNA determines the sequence of amino acids (translation) in the protein formed. Both messenger RNA (mRNA) and tRNA are produced from the DNA template. The synthesis of each protein is controlled by a different mRNA. As the peptide chain is formed, an empty space cannot be formed, which limits the peptide chain formation and protein synthesis. All 20 amino acids are needed for protein synthesis. For example, lack of essential amino acid in the diet can stop peptide chain formation and protein synthesis and affect body weight gain and animal performance.
Protein synthesis needs nucleic acids such as DNA and RNA.
Protein turnover is a dynamic process involving continuous and simultaneous protein synthesis and protein degradation. The net rate of protein gain or loss is governed by the balance of synthesis and degenerative processes. Constant turnovers of proteins in the body and the loss of proteins, mainly in faeces, are the basis for protein requirement. Even when an animal is not growing, it still has a protein requirement. The amount of protein needed in the diet depends on age, physiological (for example, pregnancy, lactation) and pathological status, and quality of the protein supplied.
Case study
A 7-year-old Quarter Horse presents to the clinic with symptoms of muscle cramping and tying-up, particularly after exercise. The owner reports that the horse has been experiencing episodes of stiffness and reluctance to move, especially after periods of rest following exercise. During the physical examination, the horse exhibits muscle stiffness and discomfort. Blood tests reveal elevated levels of muscle enzymes, indicating muscle damage. Genetic testing confirms a mutation in the GYS1 (glycogen synthase) gene, which is indicative of Polysaccharide Storage Myopathy (PSSM). Based on the clinical presentation and laboratory findings, the diagnosis of PSSM is confirmed. This glycogen storage disorder is characterised by the abnormal accumulation of glycogen in muscle tissue. There are two forms of PSSM: Type 1, caused by a mutation in the GYS1 gene, and Type 2, which also results in abnormal glycogen accumulation but without the GYS1 mutation. The primary clinical sign of this disease is muscle cramping or tying-up, though the severity and specific symptoms can vary among different horse breeds.
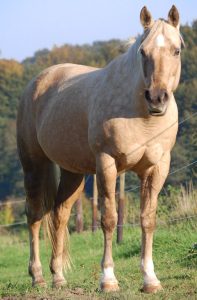
Knowledge check